Rachael Orben PhD, PI Seabird Oceanography Lab
Happy World Penguin Day (officially April 25th)! I have been contemplating what to write for my tern at the GEMM lab blog. Most of my ideas were a little bit dark, but happily when I loaded my Twitter feed Saturday morning I was greeted with many beautiful photos of penguins and the hashtag #WorldPenguinDay so that inspired something more light hearted.
To be fair, it really should be Alcidae vs. Spheniscidae (scientific family names for auks and penguins). However, I have spent many months in the field studying murres (an alcid), and I find them fascinating. Soon it will be time for them to lay their eggs at colonies along the Oregon coast, including Yaquina Head. Murres have some amazing life history characteristics.






Some of the flamboyant alcid species found in the North Pacific. These species are all crevice or burrow nesters like some penguins including Magellanic, African, and little blue penguins.
So how do murres stack up against penguins?


At first glance, murres and penguins are fairly similar. They are deep diving seabirds that forage on crustaceans and forage fish. Like murres, penguins have countershading, with black feathers on their backs and white feathers on their front. This coloring is thought to help provide camouflage when they are foraging (Cairns 1986).
There are two species of murres: common murres and thick-billed murres. Both species have a circumpolar distribution in the northern hemisphere with thick-billed murres nesting a colonies in the Arctic and common murres nesting in more temperate latitudes as far south as the central California coast. Their distributions overlap in the subarctic where they often share colonies (Irons et al. 2008).


Movement
I am under the impression that one of the reasons people love penguins so much is because they waddle. Murres aren’t so graceful either, but they spend much less of their time walking around since they commute between the sea and their colonies by flying. However, murres have to work harder to fly than they do to dive (Elliott et al. 2013). This is because they have high wing-loading. Essentially, they have big bodies and relatively small wings that they use for flying through air and water. Bigger wings would be better for air, but smaller wings are better for moving through water.
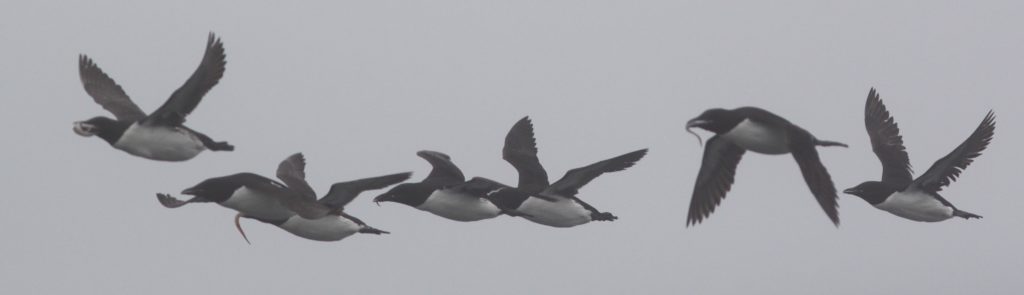
It really gets interesting when we start comparing the diving ability of alcids and penguins. Murres are the largest alcid species, and as dive depth scales with body size, they can dive the deepest. If we control for body size, alcids dive deeper then penguins (Burger 1991)! For instance, the deepest depth recorded from a thick-billed murres is 210 meters and the deepest dive of the smallest penguin (just a few hundred grams larger then the typical murre at ~1.5 kg), the little blue penguin, is a mere 69 meters (Penguiness.net).
Colonies & Nests
Murres typically nest in colonies on cliffs, off-shore sea stacks, and occasionally low lying predator free islands. Common murres use wider ledges and nest in very close proximity to each other while thick-billed murres prefer narrow ledges. Murres don’t build nests and simply lay their eggs on the rock ledge.
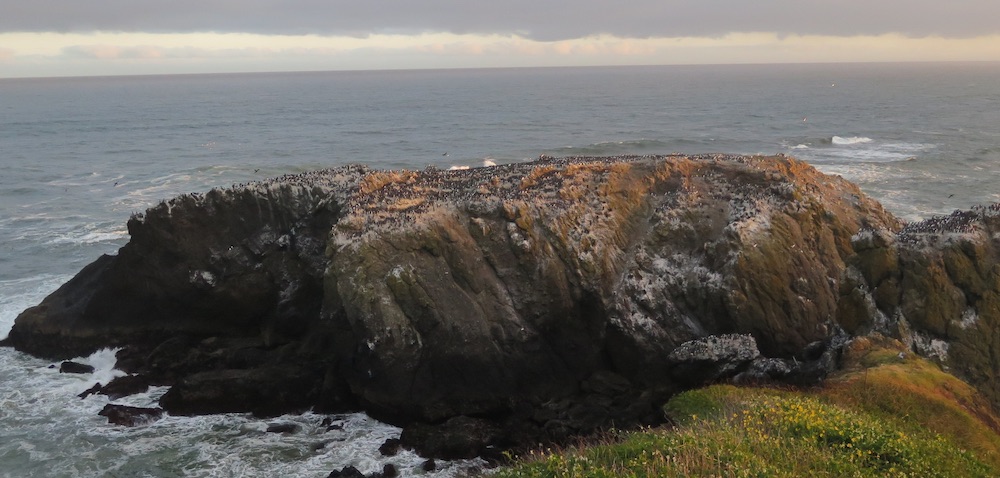
Penguin nesting colonies can take a variety of forms. Colonies of the “brush-tailed” penguins (chinstrap, Adélie and gentoo penguins) are found in places that are snow free for most of the summer. These colonies tend to form as a meandering collection of sub-colonies. These species build nests out of small rocks that they diligently collect. The rocks help keep their eggs out of snow meltwater. Emperor and king penguins stand together in a group. Burrow nesting penguins like Magellanic penguins can spread their colonies out across large areas where there is suitable habitat for burrowing.
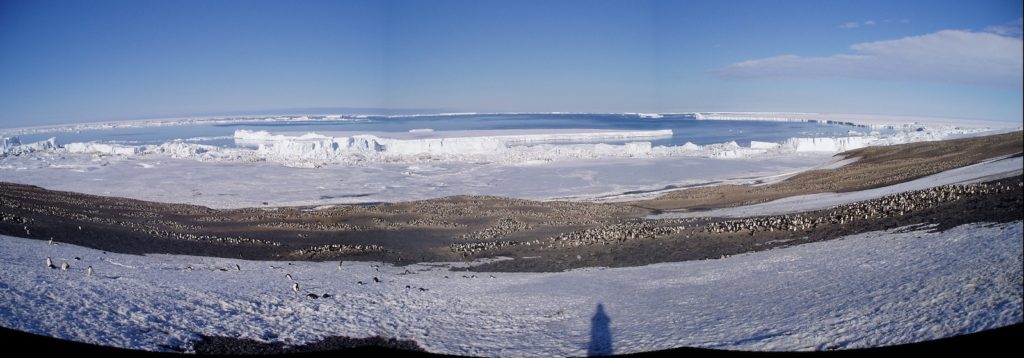
Eggs
Murres lay one large pyriform (pear-shaped) speckled egg that ranges in color from pale cream to brilliant turquoise. This variation allows them to recognize their own eggs (Gaston et al 1993)! The purpose of the shape of murre eggs is something that has been continually puzzled over, but the shape appears to help the blunt end stay cleaner, is stronger, and is more stable on sloping surfaces (Birkhead et al. 2017, 2018).
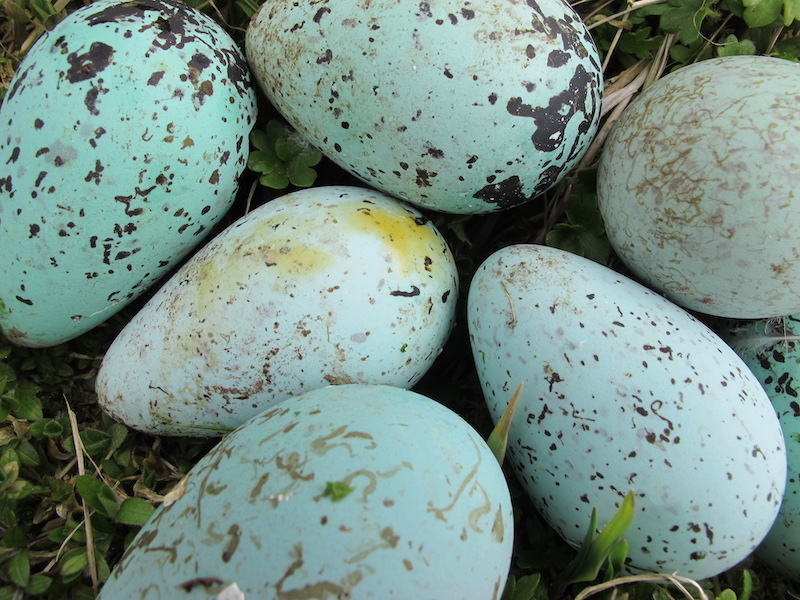
In comparison, penguin eggs don’t look that remarkable. Many penguin species lay two eggs (e.g. Adélie, chinstrap, rockhopper, gentoo), but king penguins and emperor penguins will just lay one, incubating it on top of their feet. The first egg that macaroni penguins lay is 55-75% smaller than their second egg, potentially due to constraints imposed by migration (Crossin et al. 2010).



Song
Seabirds are not generally known for their melodious songs, but they are still an important part of their social lives. For this comparison I recommend an exploration of the Cornell Lab of Ornithology’s Macaulay Library. Start with the murres and then explore some penguin species. Recently it was discovered that penguins make short noises underwater (Thiebault 2019). Perhaps murres do as well.
If you are interesting a hearing a seabird that can sing, search for Light Mantled Sooty Albatross.



Parent-Offspring Relationship
Murres bring whole fish back to the colony to feed their chick. One fish for each trip. Murre chicks fledge before their flight feathers are fully grown. They jump from the cliffs and glide down to the ocean (hopefully) where they are joined by their male parent. Then the pair paddle out to find good foraging grounds. The male parent needs to feed the growing chick frequently and by bringing the chick to the food is able to meet these demands.



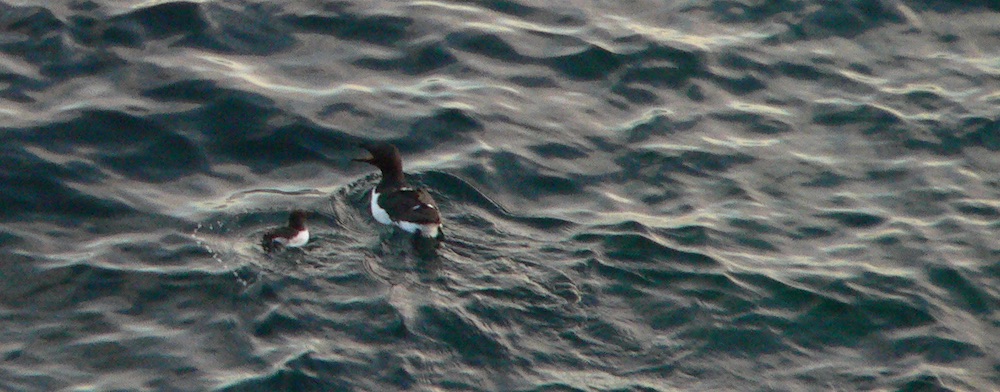
In contrast, penguins regurgitate their stomach contents to feed their offspring. They are able to carry large amounts of food this way. For instance a chinstrap penguin might bring back ~610 grams of food, almost 15% of its body weight (Miller et al. 2010). Adult penguins still have to balance their needs and the demands of their growing chicks. So the adults will leave their chicks alone once they are large enough. The chicks stand in groups known as créches to help protect them against predators like skuas.


Molt
Feather molt is an important part of all birds’ life histories. Feathers don’t last forever and need to be replaced. Both murres and penguins have unique strategies for replacing their feathers. For any flighted bird, replacing primary feathers is especially important. Murres become flightless during molt, which happens in the fall (Birkhead & Taylor 1977). This is actually thought to help their diving as with smaller wings they should be able to fly underwater more easily (Thompson et al. 1998). They replace their body feathers gradually to maintain waterproofing and warmth.
Penguins have solved this problem in another way. Instead of gradually replacing their feathers they undergo a “catastrophic molt” and replace all their feathers at once. Penguins need to be out of the water during this time and will fast, so it is advantageous to quickly grow a new coat of feathers. They too molt after their chicks are fledged.



I will let you decide which seabirds you find most fascinating, because really I find them all amazing and in need of our continued protection. Thanks for reading!
References
Birkhead TR, Taylor AM (1977) Moult of the Guillemot Uria aalge. Ibis 119:80–85
Birkhead TR, Thompson JE, Jackson D, Biggins JD (2017) The point of a Guillemot’s egg. Ibis 159:255–265
Burger, A. E. (1991). Maximum diving depths and underwater foraging in alcids and penguins. In Studies of High-Latitude Seabirds. 1. Behavioural, Energetic and Oceanographic Aspects of Seabird Feeding Ecology (ed. W. A. Montevecchi and A. J. Gaston), pp. 9-15. Canada: Canadian Wildlife Service Occasional Paper.
Crossin GT, Trathan PN, Phillips RA, Dawson A, Le Bouard F, Williams TD (2010) A Carryover Effect of Migration Underlies Individual Variation in Reproductive Readiness and Extreme Egg Size Dimorphism in Macaroni Penguins. Am Nat 176:357–366
Elliott KH, Ricklefs RE, Gaston AJ, Hatch SA, John R Speakmane F, Davoren GK (2013) High flight costs, but low dive costs, in auks support the biomechanical hypothesis for flightlessness in penguins. PNAS:9380–9384
Irons DB, Anker-Nilssen T, Gaston AJ, Byrd GV, Falk K, Gilchrist G, Hario M, Hjernquist M, Krasnov YV, Mosbech A, Olsen B, Petersen A, Reid JB, Robertson GJ, Strøm H, Wohl KD (2008) Fluctuations in circumpolar seabird populations linked to climate oscillations. Global Change Biology 14:1455–1463
Miller AK, Kappes MA, Trivelpiece SG, Trivelpiece WZ (2010) Foraging-Niche Separation of Breeding Gentoo and Chinstrap Penguins, South Shetland Islands, Antarctica. The Condor 112:683–695
Thiebault A (2019) First evidence of underwater vocalizations in hunting penguins. PeerJ:1–16
Thompson CW, Wilson ML, Melvin EF, Pierce DJ (1998) An unusual sequence of flight-feather molt in Common Murres and its evolutionary implications. The Auk 115:653–669