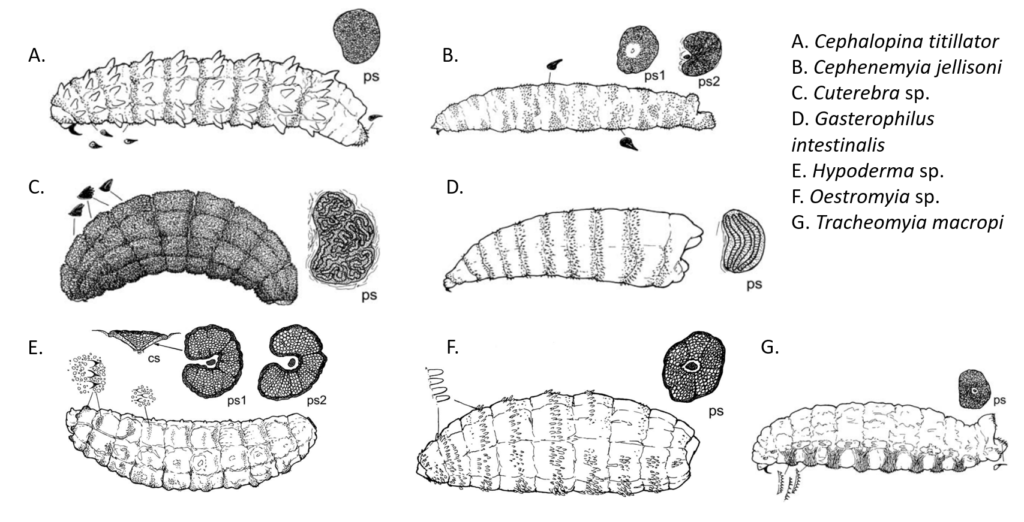
Cuticle structure, composition, & host immunity
The integumentary system (cuticle and epidermal cells) protects insects from their external environments, contributes to the maintenance of homeostasis, and plays a role in other important processes, including respiration and mobility. For endoparasites such as bot fly larvae, the cuticle, or exoskeleton, is the largest organ exposed to the host organism’s inflammatory and immune responses and, as such, provides a first line of defense against them [1,2]. In the horse stomach bot, Gastrophilus intestinalis (Diptera: Oestridae), the quantity of extractable proteins in the cuticle decreases between the larval and pupal stages due to reduced solubility following sclerotization; as in most other holometabolous insects, bot fly larvae possess a relatively soft, unsclerotized cuticle [3]. Compared to non-parasitic fly larvae, such as Drosophila melanogaster, the Old World skin bots, Hypoderma spp., have been reported to develop thicker pro- and inner epicuticles as they grow, suggesting that the structure and composition of the integument exhibits adaptations to their developmental environment [2].
While the structure and composition of the integument has not been investigated for all species, it has been characterized for the sheep nose bot, Oestrus ovis [1, 4]. Like other insects, O. ovis larvae possess a basement membrane, layer of epidermal cells, and overlying cuticle, which differentiates into procuticlar and epicuticlar layers during the third instar [4]. Quinones are thought to stabilize lipoproteins in the inner epicuticle and cuticulin layer, strengthening the structure [4]. Tubular structures that appear to be wax canals have been observed in O. ovis and Hypoderma spp., suggesting that a wax layer may be secreted, providing a minimal level of protection from desiccation while the larvae travel to the site of initial penetration [2, 4]. In immunoblotting experiments, most extracts from O. ovis larval cuticles elicited only weak immune responses in serum from host organisms (sheep and rabbits), although some high molecular weight polypeptides acted as immunogens; the insoluble epicuticular layer may prevent the host immune system from accessing these immunogenic proteins [4].
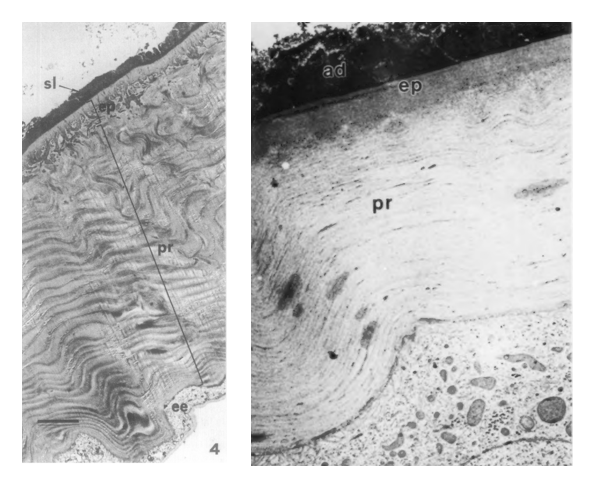
Some work has also been done to investigate the role of the cuticle in allowing Hypoderma spp. to evade the immune responses of their hosts. The outer cuticular layer of first instar Hypoderma lineatum larvae appears to contain negatively-charged molecules, perhaps acid mucopolysaccharides [2]. Some pathogenic nematodes and bacteria also exhibit negatively-charged outer layers that are thought to reduce hemocyte affinity for the cell surface, mask immunogenic proteins, and minimize inflammatory responses in the host that can be lethal to the pathogen; a similar mechanism may help hypodermatid larvae avoid activating the host immune system as they burrow through the upper dermal tissue to their development sites [2, 5]. Biochemical countermeasures also contribute to larval immune-evasion: Hypoderma spp. secrete serine proteases that inhibit production and responsiveness of host lymphocytes and peripheral blood mononuclear cells [6]. The cuticular composition of the larvae of bot fly species other than O. ovis, H. lineatum, and H. bovis has not been studied extensively [1].
Attachment, migration, and feeding on the host
Sclerotized structures help bot fly larvae to feed, attach to host tissues, and secure their position within the development site [1, 7]. Mouth hooks, which are used to scrape host tissues during feeding and aid in attachment to the host body, exhibit morphological diversity among bot fly subfamilies [7, 8]. In Cuterebrinae and Oestrinae, the hooks curve and taper gently and point downward relative to the body, while in most Gastrophilinae, the hooks curve abruptly and point upward [8]. Following initial introduction into the host animal’s mouth as a first instar larva, the strongly curved mouth hooks of Gasterophilus intestinalis help it to attach within the oral cavity so that it can burrow into the interdental space where it completes its first-to-second instar molt prior to traveling into the stomach [9]. The mouth hooks in Hypodermatinae are generally smaller than in other subfamilies but increase in size in later instars to aid the larva in scraping cells from the warble surface [1,8]. The direction, shape, and prominence of mouth hooks in larvae of different species is thought to be adaptive for different entry and migration routes within the host body; therefore, similarity in mouth hook morphology may imply similar migration routes, while unique morphology suggests a unique migratory route or mode of locomotion [8].
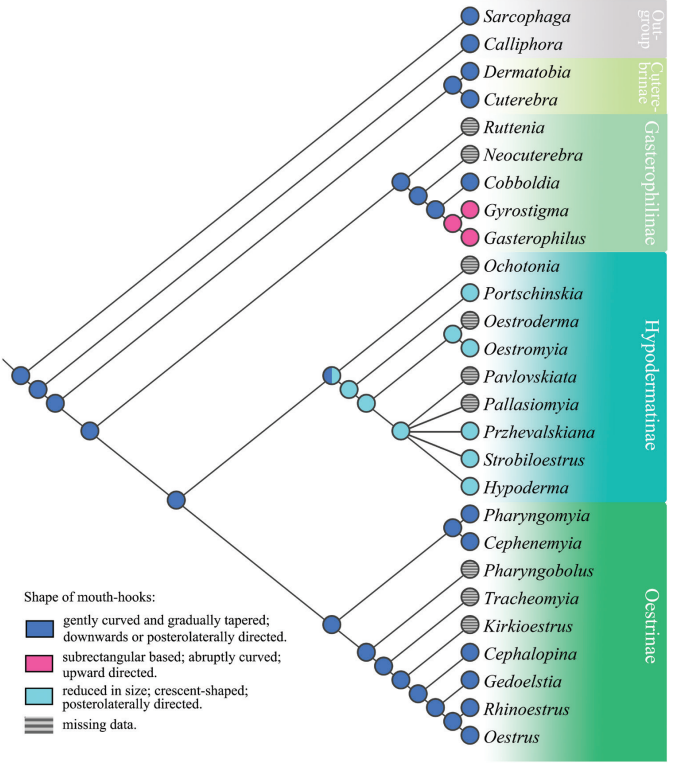
Similarly, the position, arrangement, and prominence of cuticular spines may reflect different larval attachment and migration strategies. The spines on Oestrinae (nose bots) are concentrated on the ventral and lateral portions of the body, facilitating movement over mucosal surfaces after the larvae are deposited onto the host by the larviporous female [1]. Hypodermatinae, which burrow into host skin, exhibit uniformly distributed, relatively sparse spines oriented toward the posterior of the body; this arrangement is likely adaptive for anchoring the larva within the warble and assisting in nutrient acquisition by scraping the host cells in the walls of the cyst [1]. Cuterebra and Gasterophilinae generally possess bands of spines that are reduced on the terminal segments of the abdomen [1, 10]. In Cuterebra, the backward orientation of the spines may help to maintain larval position within the warble. Larvae in this subfamily also possess a terminal sac that facilitates movement over the host body prior to burrowing into the skin at the development site [1].
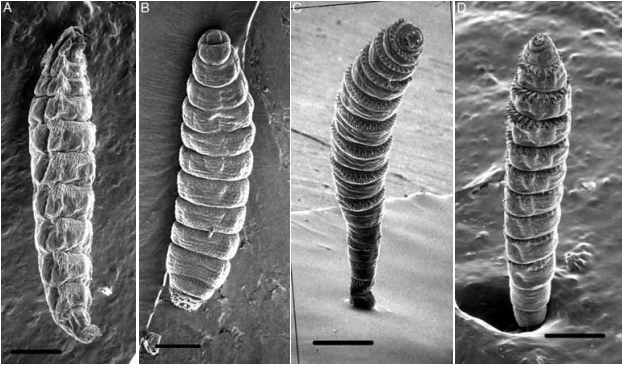
References:
[1] Cowell, D.D., et al. Oestrid Flies : Biology, Host-Parasite Relationships, Impact and Management, CABI, 2006. ProQuest Ebook Central, https://ebookcentral.proquest.com/lib/osu/detail.action?docID=289452.
[2] Colwell, D. D. (1991). Ultrastructure of the Integument of First-Instar Hypoderma lineatum and H. bovis (Diptera: Oestridae). Journal of Medical Entomology, 28(1), 86–94. https://doi.org/10.1093/jmedent/28.1.86
[3] Yousef, H. A. (2004). CHARACTERIZATION OF CUTICULAR PROTEINS OF THE HORSE BOT FLY, GASTROPHILUS INTESTINALIS DURING LARVAL-PUPAL DEVELOPMENT. Efflatounia, 4, 41–48.
[4] Innocenti, L., Lucchesi, P., & Giorgi, F. (1997). Integument ultrastructure of Oestrus ovis (L.) (Diptera: Oestridae) larvae: Host immune response to various cuticular components. International Journal for Parasitology, 27(5), 495–506. https://doi.org/10.1016/S0020-7519(96)00186-5.
[5] Wang, C., & St. Leger, R. J. (2006). A collagenous protective coat enables Metarhizium anisopliae to evade insect immune responses. PNAS, 103(17), 6647–6652. https://doi.org/10.1073/pnas.0601951103
[6] Otranto, D. (2001). The immunology of myiasis: Parasite survival and host defense strategies. Trends in Parasitology, 17(4), 176–182. https://doi.org/10.1016/S1471-4922(00)01943-7
[7] Scholl, P. J., Colwell, D. D., & Cepeda-Palacios, R. (2019). Myiasis (Muscoidea, Oestroidea). In Medical and Veterinary Entomology (3rd ed., pp. 383–419). Academic Press. https://doi.org/10.1016/B978-0-12-814043-7.00019-4
[8] Li, X. Y., Pape, T., Colwell, D., Dewhurst, C., & Zhang, D. (2021). Three-dimensional characterization of first instar horse and rhinoceros stomach bot fly larvae (Oestridae: Gasterophilinae: Gasterophilus, Gyrostigma): Novel morphology and evolutionary implications. Zoological Journal of the Linnean Society, 193, 939–952. https://doi.org/10.1093/zoolinnean/zlaa164.
[9] Cogley, T. P., Anderson, J. R., & Cogley, L. J. (1982). Migration of Gasterophilus intestinalis larvae (Diptera: Gasterophilidae) in the equine oral cavity. International Journal for Parasitology, 12(5), 473–480. https://doi.org/10.1016/0020-7519(82)90079-0
[10] De Filippis, T., & Leite, A. C. R. (1998). Morphology of the second- and third-instar larvae of Dermatobia hominis by scanning electron microscopy. Medical and Veterinary Entomology, 12, 160–168. https://doi.org/10.1046/j.1365-2915.1998.00096.x.